Deepwater, high-pressure completion tubular maximizes combined-load capabilities
Comprehensive FEA, structural/sealability testing program conducted
By T. Anderson, C. Fontenot and R. Davey, Chevron; J. Dugas and B. White, Quail Tools LP; K.A. Hamilton,
C-FER Technologies; P.S. Beauchamp, L.C. Karlapalem, A. Muradov and J.N. Brock, NOV Grant Prideco
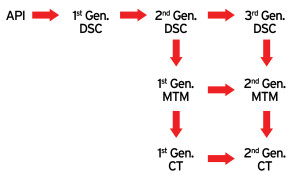
This article discusses a two-year comprehensive effort to design, test, manufacture and deploy a new high-pressure completion tubular for Chevron’s deepwater Gulf of Mexico operations. The major challenge was to design, test and manufacture a subsea completion string that would provide efficient hydraulics during the fracturing operations while ensuring mechanical and pressure integrity.
Rotary-shouldered connections
Since the industry first began rotary drilling, wells have continued to become more challenging. Drilling tubulars have evolved as well, enabling operators to meet these challenges. The development of rotary-shouldered connections (RSCs) has been a key enabling technology by providing the mechanical and hydraulic performance required to drill more difficult and complex wells.
First-generation double-shouldered connections (1st Gen. DSCs) incorporate the same basic design features, such as thread form, taper, lead and pitch diameters, as the API RSC connections, but they include a secondary torque shoulder added inside the box member at the pin nose interface. These connections increased the connection torsional strength by approximately 40% over the corresponding API connection with the same outside diameter (OD) and inside diameter (ID).
The increased torsional strength provided by the secondary shoulder allowed designers to streamline the connection, increasing the ID and/or decreasing the OD, in order to improve the hydraulic performance of the drill string.
To develop the 2nd Gen. DSCs, changes to the standard API RSC design parameters were necessary. Enhanced thread forms with lower stress concentrations and shallower tapers to increase the area of the internal torque shoulder were necessary to increase torsional performance and further streamline connection dimensions. The 2nd Gen. DSC provides approximately 25-30% more working torque capacity, or an improvement in working torque of approximately 65-70%, compared with a standard API RSC of similar dimensions. The 2nd Gen. DSC has streamlined connection dimensions, enabling one pipe size larger to be run in the same hole size, significantly improving hydraulic efficiency while maintaining the ability to fish the connection with an equivalent overshot.
One of the primary philosophies employed during the development of 3rd Gen. DSC was the concept of “one size does not fit all.” Common drill pipe sizes that were employed throughout the connection development program were grouped into four categories. Design requirements for each group were prioritized, and four connection design configurations were developed to meet the specific and needs of each drill pipe size.
Compared with the 1st and 2nd Gen. DSCs, the 3rd Gen. DSC had a dual-start, twin-lead or double-start thread. Double-start threads incorporate two threads spaced 180° apart, reducing the number of turns to make up the connection by 50%. Further, the 3rd Gen. DSC design incorporated a dual-radius thread form that reduced peak stresses and enhanced fatigue resistance.
Tapers were reviewed for each connection size and optimized to balance the needs of the specific drill pipe size. Compared with the 2nd Gen. DSC, connection sizes prioritizing speed of makeup resulted in increased tapers, and connection sizes prioritizing increased torsional capacity resulted in equivalent or reduced tapers. Similar to taper, thread pitch was reviewed for each connection. Connection sizes prioritizing speed of makeup resulted in faster thread pitch to minimize the number of turns from stab to makeup. Also, API tool joints are produced with specified minimum yield strength (SMYS) of 120,000 psi, while 3rd Gen. DSCs utilize higher strength (130,000 psi SMYS) material to improve torsional capacity and/or provide more streamlined dimensions for hydraulic efficiency.
Gas-tight RSCs
After the introduction of the 2nd Gen. DSC in 1998, a project was initiated to develop the first RSC with the ability to seal high gas pressures. The first-generation gas-tight (metal-to-metal seal) rotary-shouldered connection (1st Gen. MTM) utilized the reduced thread height and flattened taper of the 2nd Gen. DSC design to incorporate a radial metal-to-metal interference seal on the pin nose.
With the primary seal being the radial metal-to-metal seal on the pin nose, the external shoulder provides a secondary seal. The internal shoulder acts as a torque stop and is not considered a pressure seal. This permits the connection to be racked back on the pin nose during tripping operations without concern about damaging a seal.
Interference is one of the controlling factors in the effectiveness of a radial metal-to-metal seal. The primary importance was optimizing the seal interference in order to ensure adequate contact stresses at the sealing surfaces. Insufficient interference would adversely affect the connection sealability, while excessive interference could cause galling. The 1st Gen. MTM connections provided 15,000-psi internal pressure and 10,000-psi external pressure ratings.
The addition of a gas-tight seal to the connection permits safe high-pressure drill stem testing (DST) operations using the drill pipe. Consequently, a single string of drill pipe can be used for drilling operations, workover and completion operations, and drill stem testing. The connection handles and makes-up like a conventional API RSC and resists galling after multiple make and break cycles. It also provides pressure sealing like that of a typical premium tubing connection.
The objective while developing a 2nd Gen. MTM was to improve the pressure ratings and incorporate the superior performance and running and handling benefits of the 3rd Gen. DSC. Design requirements for the 2nd Gen. MTM were defined as 20,000-psi internal and 10,000-psi external pressure – a 5,000-psi increase over the 1st Gen. MTM internal pressure rating.
Completion tubular requirements
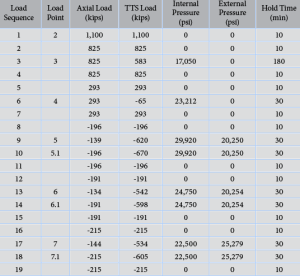
In March 2002, Chevron drilled the Tahiti #1 well in 4,017 ft of water to a total depth of 28,411 ft. Two appraisal wells were then drilled simultaneously in Green Canyon blocks 596 and 640. The appraisal program verified Chevron’s initial estimates of 400 million to 500 million barrels of ultimate recoverable oil reserves. To complete the discovery well, several complex challenges faced the Tahiti Well Test Team.
Due to the extreme depth and bottomhole pressure of the Tahiti reservoir, the completion and well test required the design of numerous new equipment to perforate, fracpac and flow test the Tahiti #1 discovery well, including the design and qualification of perforating and fracpac completion tubular. Requirements for the completion tubular included:
• Fishable within the 9 7/8-in. tieback, limiting the connection OD to 7 in.;
• An ID of no less than 4 1/4-in. to provide the desired treating rate during fracturing operations;
• An approximate 29,000-psi absolute internal working pressure rating to sustain internal pressure and not leak, potentially jeopardizing the fracpac results;
• An approximate 24,500-psi absolute external working pressure rating to sustain elevated annulus pressures and not collapse or leak;
• Withstand multiple make-up and breakout cycles while maintaining sealability at pressure to enable multiple trips into the well for cleanout, displacement, perforating and fracture operations; and
• A high-performance metal-to-metal seal that possessed integrity against fluid leak.
A vertically integrated, comprehensive design and qualification program was commissioned for the completion string – the first-generation built-for-purpose completion tubular (1st Gen. CT). The 1st Gen. MTM connection served as the basis of the 1st Gen. CT connection. Finite element analysis (FEA) of the 1st Gen. MTM revealed that the extended box counterbore lacked stability under the immense external pressure and compressive loads anticipated at the bottom of the well. Sectional stresses in the box counterbore significantly exceeded the material capabilities, and counterbore collapse was predicted.
To provide additional rigidity and structural stability, the counterbore length was shortened from 2 1/4 in. to 5/8 in. Adjusting the box counterbore length created an imbalance of forces acting on the internal and external shoulders. The initial clearance, or gap, between the pin nose and the box internal shoulder when the connection is made-up “hand-tight” and the make-up position when the external shoulder and thread flanks are just touching, had to be determined. The proper gap for the 1st Gen. CT was determined through a series of FEA runs.
The new design was then analyzed with FEA against the anticipated Tahiti loads. The connection showed that sectional stress values in the box counterbore still exceeded the 120,000-psi tool joint material SMYS. A decision was made to incorporate 135,000 psi SMYS tool joint material for the connection.
Rigorous physical testing verified the design, and the 1st Gen. CT was deployed on the Tahiti #1 well.
2nd Gen. CT
The objective of the new deepwater, high-pressure completion tubular was to maintain or improve the combined-load capability of the 1st Gen. CT while providing the speed of makeup and proven cost savings of the 3rd Gen. DSC.
The 1st Gen. CT benefits from the refinement and optimization of the 3rd Gen. DSC design. The design requirements were met through the combination of high-strength material, proven radial metal-to-metal seal technology and the double-start thread, which reduced the number of turns necessary to assemble the connection by 50%. Other design parameters of the 2nd Gen. CT included dual-radius thread form, which enhances connection fatigue performance, and optimized taper, pitch and thread height. These are identical to those found on the 3rd Gen. DSC. The verification of the 2nd Gen. CT design involved FEA and a rigorous physical test program.
Higher tool joint yield strength allowed the optimization of the connection’s taper and thread height to increase stabbing depth, which reduced the number of turns from stab to makeup compared with the 1st Gen. CT. The deeper stab depth provides increased stability as the pin connection is first rotated, which reduces the chances of cross-threading. Additionally, deeper stab reduces the likelihood of creating pinch points, increasing operator safety.
Comprehensive FEA
A comprehensive FEA was conducted to optimize the design and evaluate the performance of the 2nd Gen. CT connection. A 2D axisymmetric model of the connection was generated using commercial software. Non-linear material properties were used to model the 135,000-psi SMYS tool joint material and 150,000-psi SMYS tube material. Adaptive mesh was used for the connection model with the finer mesh elements present at the thread roots and other conventional regions of stress buildup. All effects of bending loads and elevated temperatures were ignored in the numerical model.
The extreme values of pin length and box depth were calculated based on manufacturing tolerances. These were then used to compute the working range for the initial clearance at the internal shoulder. Similarly, the working range for the interference at the radial metal-to-metal seal was computed based on the manufacturing tolerances. Thus, four distinct configurations were evaluated:
• Maximum internal shoulder gap with maximum seal interference;
• Maximum internal shoulder gap with minimum seal interference;
• Minimum internal shoulder gap with maximum seal interference; and
• Minimum internal shoulder gap with minimum seal interference.
Axial interference was used to simulate the torsional makeup of the connection. Contact forces at the external and internal shoulders, seal and threads were calculated and used to compute the applied torque to the connection. A coefficient of friction of 0.08 was assumed at all contacting surfaces.
When the makeup torque is applied to the connection and both shoulders are engaged, the region between the external shoulder and the radial seal and that between the radial seal and the internal shoulder are assumed to be at atmospheric pressure. Simultaneous application of internal and external pressures on a tubular, even when equal in magnitude, results in both tangential and radial stresses. Hence, the absolute external and internal pressures as shown in Table 1 were applied on the connection OD and ID, respectively.
Among all the load points analyzed for all four design configurations, the worst-case stress distribution was observed in the box counterbore region of the maximum internal shoulder gap with maximum seal interference configuration under applied recommended makeup torque and service load point 7.1. However, the sectional stress values in this region were within the SMYS limits.
Based on the FEA results, the 7-in. OD x 4 1/4 in. ID 2nd Gen. CT connection displayed acceptable stress profiles for all the service load points listed in Table 1.
Connection testing
Comprehensive full-scale physical testing of the 2nd Gen. CT connection design was necessary to verify performance for the operating conditions expected in HPHT offshore wells. The testing program consisted of two phases: (1) galling resistance (make and break) testing, and (2) structural/sealability testing. The make and break testing subjected the candidate connection samples to multiple makeup and breakout cycles to assess the galling resistance of the connection. This was performed at the manufacturer’s facilities in Houston. The structural/sealability testing subjected the same connection samples to a range of combined loading conditions, including axial tension and compression loads, simultaneous internal and external pressures, elevated temperature and bending loads. The structural/sealability tests were performed by C-FER Technologies at its laboratory in Edmonton, Alberta.
Connection samples
Three 2nd Gen. CT connection samples were machined from 7 1/2-in. diameter 4130M bar stock material that was quenched and tempered to achieve minimum yield strength of 135,000 psi. Each of the three samples was machined to meet specific critical tolerance configurations:
• Sample 1 – Maximum internal shoulder gap with maximum seal interference (worst-case configuration for box counterbore stress).
• Sample 2 – Minimum internal shoulder gap with maximum seal interference (worst-case configuration for pin nose stress).
• Sample 3 – Maximum internal shoulder gap with minimum seal interference (worst-case configuration for connection sealability).
An additional sample was machined from the same material for each tolerance configuration in case the original samples were damaged at any point in the testing process.
Make and break testing
The make and break test program subjected the connection samples to 23 makeup and breakout cycles wherein the samples were made up to 64,100 ft-lb, the maximum recommended makeup torque adjusted by the copper-based thread compound’s specified friction factor of 1.15. This testing was performed to simulate the manufacturing break-in process and the anticipated maximum number of make and break cycles that the connection would encounter in service. The make and break cycles were performed using continuous torque-turn equipment and monitoring system that rotated the pin end of the connection into the box end until the desired makeup torque was achieved. The connections were visually inspected intermittently to identify whether any damage had occurred.
Prior to the 23rd makeup of Sample 3, two small bypass holes were drilled – one through the box counterbore and one through the pin nose. These holes were drilled to prevent the external shoulder and internal shoulder from sealing during the structural/sealability test program. Disabling these seals focused the sealing performance on the radial metal-to-metal seal during the test. Neither Samples 1 nor 2 had similar holes drilled as they were tested to assess the performance of the connection design as a whole rather than the sealability of the radial metal-to-metal seal.
Program preparation
After the samples arrived at the test laboratory, they were broken out and cleaned so that biaxial strain gauges could be applied to the inner bore of the pin nose. Biaxial strain gauges were also placed on the box end OD adjacent to the external shoulder of the connection, as well as on the external pipe body surface of the pin-end of the connection samples to monitor pipe body curvature during the test. Four strain gauges were placed 90° circumferentially from one another at these locations for 12 strain gauges per sample.
Once the strain gauges were affixed and their high-pressure-resistant jacketing applied, the connection samples were cleaned and inspected for damage. After applying thread compound to the pin and box connections, final makeups were performed with a ratcheting-style hydraulic power makeup system that rotated the pin end of the connection into the box end in 30° increments until the desired final makeup torque was achieved. Samples 1 and 2 were made-up to 64,100 ft-lb, and Sample 3 was made up to the minimum recommended make-up torque of 53,400 ft-lb.
The strain gauges around the connection of each sample were connected to a data acquisition system to record the strain during makeup. Maximum recommended makeup torque provided the worst case for the connection’s counterbore stress and the pin nose stress for Samples 1 and 2, respectively. Minimum recommended makeup torque provided the worst case for the connection’s sealability for Sample 3.
Program load points
The structural/sealability test program load points were designed to replicate various load combinations that the connections could be subjected to during installation and operation in offshore HPHT applications. The load points for this program subjected the connection samples to net axial loads between 1,100 kip in tension and 215 kip in compression, temperature up to 220°F, bending loads to create a 3°/100 ft dogleg severity (DLS), and internal and external pressures up to 29,920 and 25,279 psi, respectively. The three load points (5.1, 6.1, and 7.1) were used to compensate for the axial tensile load as a result of the capped end pressure effect created by the external geometry of the samples when they were subjected to external pressure. The load points for each connection sample are listed in Table 1.
The load points were repeated over three cycles. The first and third cycle load points were performed in the sequence shown in the table and at 220°F. The second cycle load points were performed in reverse order at ambient temperature. A customized external pressure vessel incorporating proprietary sealing mechanisms to allow free bending of the sample was used to apply external pressure to the samples while they were subjected to the 3°/100 ft DLS. The dogleg curvature was applied through four-point bending, and pressure-resistant linear variable differential transformers were positioned along the external pressure vessel to directly measure the lateral displacement of the samples. The vessel’s feed-through ports allowed the strain gauges on the samples to be monitored in real time. Due to the high temperature of Cycles 1 and 3, an electrically non-conductive mineral oil was used as the pressurizing medium.
All of the load points where external pressure was applied to the connection samples had internal pressure applied simultaneously. Applying both internal and external pressure at the same time was critical in the overall evaluation of the 2nd Gen. CT connection’s performance. If just the net differential pressure had been applied, only the sealability characteristics of the radial metal-to-metal seal would have been evaluated. By applying internal and external pressures simultaneously, the test program not only determined the sealability characteristics but further established whether the connection was capable of withstanding the combined axial, radial and hoop stresses representative of those in the eventual expected operating conditions for this connection design.
Test results
The structural/sealability tests were performed between 8 March and 20 April of 2013. The samples were tested individually. Each sample was assembled with the test fixtures and external vessel making-up the test apparatus and then installed in a load frame with an axial load capacity of 3,400 kips in both tension and compression.
Since the test apparatus was constructed in such a way that no leak path was possible from the interior to the exterior pressure chambers, a pressure change between the inner bore of the sample and the external vessel could only be attributed to leakage across the sample connection. As such, connection sample sealability performance was assessed by monitoring the pressure differential across the connection. None of the three samples tested showed any signs of leakage across their connections under either a net external or internal pressure differential.
Conclusion
The objective to design, test and manufacture the 2nd Gen. CT, a new high-pressure completion tubular for Chevron’s deepwater Gulf of Mexico operations, was successfully met.
This article is based on SPE/IADC 173034, “New Deep-Water, High-Pressure Completion Tubular for Gulf of Mexico Maximizes Combined-Load Capability While Incorporating Proven Cost Saving Features,” presented at the 2015 SPE/IADC Drilling Conference, 17-19 March in London. A full version of this paper can be found at the OnePetro website.