Case studies: High-density elastic cements applied to solve HPHT challenges in South Texas
By Barry Wray, Cimarex Energy; David Bedford, Lennox Leotaud and Bill Hunter, Halliburton
Well cementing operations in South Texas tend to present a number of challenges to those responsible for constructing oil and gas wells. For instance, the temperatures and pressures at which the cement needs to be placed can be extreme, routinely exceeding bottomhole static temperatures of 300°F and pore pressures requiring fluid densities of 17 lbm/gal or greater to maintain well control. These extreme conditions can present challenges not only during placement of the cement slurry in the wellbore but also later to the set cement sheath during the life of the well.
To effectively meet these challenges, well operators in South Texas have been using high-density cements that have been mechanically modified so the set cement will be more elastic and resilient. Advanced diagnostic software is used to predict well situations where these cements are required.
Currently, high-density elastic cements (HDEC) have been placed in more than 40 wells in southern Texas, and the use of these sealants combined with diagnostic software has become routine.
This article discusses the challenges cementing high-pressure, high-temperature (HPHT) wells in South Texas, then details the best practice life-of-the-well solutions that have been applied.
BACKGROUND
South Texas has long been known for its HPHT reservoirs where drilling can prove a formidable exercise. In addition to difficulties dealing with high pressures and temperatures, low-pressure zones can be present.
In recent years, well designs have also become more complex as fields mature and operators search for new sources of oil and gas. Cementing the casing and liner strings in place in these wells is a critical phase in the well-construction process. The cement sheath is vital in that it protects and supports the casing and delivers the necessary zonal isolation for the well.
Once the well is completed, a new set of challenges that can stress an initially competent cement sheath are encountered. For example, some South Texas HPHT wells require high-pressure fracturing operations to unlock their full production potential, and in other wells, the heavy-weight drilling fluid with which the well is drilled is swapped out with lightweight brine at some point during the well completion process. In both cases, the loads imposed on the cement sheath can be extreme, potentially leading to debonding and even the catastrophic failure of a conventional cement sheath.
When this occurs, the situation generally can not be effectively remediated, and an operator might lose a costly wellbore. To address this problem, in 2006 it was identified that HDEC, designed to behave more elastically than conventional, brittle cements, can be applied to HPHT wells in the area with success. Use of HDEC has since become widespread in South Texas. Presently, HDEC have been placed for eight operators on 47 occasions in the area at temperatures up to 448°F and densities up to 18.9 lbm/gal.
WELL CONDITIONS
Many of the key plays in South Texas are HPHT, and two typical plays are the Yegua and Cook Mountain oil and gas plays. The Yegua play is defined by sandstone reservoirs that produce oil and gas from sandstones and deep, overpressured reservoirs in a band across the western Gulf of Mexico. The Cook Mountain sandstone gas play is defined by sandstone reservoirs that produce gas from structural traps across the western Gulf. The sandstones in both of these plays are generally characterized as having high permeability and porosity (Table 1).
Temperature gradients in the Yegua and Cook Mountain formations range from 1.4 to 2.1°F/100 ft. Most wells drilled into these sands come on production after being perforated with tubing-conveyed perforation (TCP) guns and flow naturally because of the high permeability of the sands.
One significant drilling challenge is insufficient borehole pressure integrity, especially when cutting through depleted zones intermingled with higher-pressure intervals. Shales and/or sands weakened by depletion, leaking faults or unfavorable rock properties result in lost returns when mud weights are close to pore and fracture pressures.
In one field, for example, oftentimes the target sand is normally pressured with overpressured shales above and below the sand, with some faulting present. Setting a casing string to isolate normal-pressure zones from high-pressure zones can be problematic if the faults exist at the casing shoe and the cement sheath does not provide a good hydraulic seal. Additional casing strings are often set to allow drilling to continue with narrow margins between pore- and fracture-pressure profiles. These narrow margins can create potential well control issues while construction of the well is in progress.
These conditions can also drive the selection process for the drilling fluids to use in these wells. Oil-based mud (OBM) systems provide definite advantages, generally delivering improved shale stability and clay control, reduced pore-pressure transmission, less differential-sticking tendencies, higher rates of penetration, and better lubricity to minimize torque and drag.
However, these fluids are often a costly solution with regard to disposal, lost-circulation issues and regulatory/environmental considerations. Therefore, when there are small differentials between pore pressure and fracture-initiation pressure, operators in South Texas prefer to use conventional water-based mud (WBM) below the intermediate casing string to better combat losses and hole ballooning, even though larger washouts are possible with WBM. Hole ballooning is defined by the walls of the well expanding outward because of the increased pressure during pumping. When pumping stops, the walls contract and return to normal size, forcing excess mud out of the wellbore.
Generally, wells drilled in fields by this operator follow a well plan commonly used throughout South Texas. Typical wells are drilled between 11,700 ft and 16,000 ft total depth (TD), with bottomhole temperatures ranging from 270°F to 340°F.
Surface casing
Surface casing is set at depths between 2,500 ft and 5,000 ft measured depth (MD), covering shallow lost-circulation and fresh-water zones. Surface casing depth and cement properties are defined by the Texas Rail Road Commission.
Intermediate casing
When drilling continues below the surface casing, higher bottomhole pressures often require a heavier mud. The heavier mud can break down weak zones, causing severe lost-circulation problems. To protect these weak zones, an intermediate string of casing is set at depths from 10,000 ft to 13,000 ft MD, just above or below the top of the nearest high-pressure formation.
The intermediate hole section can have multiple sand sections that can cause the casing to differentially stick before reaching TD. Good centralization is therefore important, as is running the casing to bottom as fast as possible, and when on bottom, not moving the casing. The intermediate string of pipe is cemented with tops of cement varying.
The top of cement (TOC) on an intermediate casing design oftentimes must allow for annular pressure to dissipate into the formation because hot mud passing up the drill pipe/casing annulus can cause fluid behind the intermediate casing to heat up and expand. This expansion has the potential to cause casing collapse; therefore, having a TOC at a depth below the previous casing is generally desired on HPHT wells.
The intermediate casing cement job typically requires an optimized spacer design and high displacement rates to maximize mud-displacement efficiency.
Production casing
On completion of the intermediate casing cement job, the drilling fluid density is increased, the well is drilled to TD, and production casing is set and cemented. The production casing cement sheath is required to effectively isolate water zones from pay sands. Sometimes a contingency drilling liner might be required in the process of drilling to TD if either a low-pressure or higher-pressure zone is encountered, compromising borehole-pressure integrity. This leads to the undesirable effect of reducing the diameter or drilling further and downsizing the well.
Operators in South Texas are implementing many new technologies as they strive to effectively exploit HPHT reservoirs. These include underbalanced drilling and drilling with casing to avoid associated problems with the intermingling of high-pressure and depleted zones, novel lost-circulation curing techniques and mechanically modified cements.
WELL INTEGRITY
The largest specific challenges these conditions create for those tasked with placing an effective cement seal in HPHT wells are:.
- High equivalent-circulating densities (ECDs) are created when the densified fluids required for well control purposes are pumped into the well, often leading to formation breakdown, followed by annular fluid loss in the fractures created that can potentially lead to well-control issues.
- After high-pressure well stimulation and production, casing failures can occur if the cement sheath that has been placed is incomplete or does not possess the requisite mechanical properties to withstand the high differential pressures to which it is subjected.
To combat the first concern, accurate pre-job dynamic-hydraulic computer simulations are important to assess whether the ECD throughout the open-hole annular area will stay below any known weak-zone fracture gradients. The narrow windows between those fracture gradients and the high mud weights can make this task particularly difficult. Obtaining the most up-to-date wellbore- and drilling fluid parameters for the simulations is imperative if a successful outcome is to be achieved. Like all computer models, the worth of the output is based on the quality of the data input into the model.
Once the wellbore definition is known, the next piece of the puzzle is the accurate modeling of fluid viscosities, one of the most prominent factors in the calculation of downhole ECDs. A variety of rheological models are available, such as Bingham plastic, power law and Hershel Bulkley. Each model has a different effect on the ECD. Choosing the right model is not as easy as requesting a specific plastic viscosity (PV) and yield point (YP), the two parameters specifically associated with the Bingham plastic model.
Methods are available today that will convert rheometer data into the best-fit model for any specific situation, enhancing the way downhole ECDs are calculated and helping lower the chance of losses caused by inadvertently fracturing the open hole during drilling and cementing operations.
Traditionally, the success or failure of a primary cement job was judged on its capability to safely place the designated volume of cement at a predefined depth in the annulus. Cement bond logs have also long been available as a tool with which operators can make an initial assessment of the bond between the cement sheath and the casing string.
However, the industry is now beginning to recognize that the success of a cement job should be based on the cement sheath’s ability to maintain zonal isolation throughout the life of the well. In the HPHT wells of South Texas, the set cement sheath is subjected to an extreme range of temperatures and pressures through completion, fracture stimulation and production cycles. Considering most of these wells are drilled with heavy-weight drilling fluid and cemented with even denser slurries, the pressure drop experienced inside the casing when swapping out to the much lighter completion fluids used can create a large amount of stress on the cement-casing bond, potentially leading to debonding issues.
In a number of cases, wells might also be fracture-stimulated directly through the cemented casing, creating a large cooling effect on the cement sheath combined with a large increase in pressure. These extreme conditions have led to several casing failures in South Texas because of the inability of conventional, heavy-weight cement to maintain its integrity during these cycles, often resulting in debonding from the casing or shear deterioration in the cement sheath. Once it has failed in this manner, the cement sheath might no longer provide zonal isolation; instead, it can act as a conduit for formation fluids to apply pressure to the casing, ultimately resulting in casing collapse.

For example, Figure 1 shows the results of an analysis performed on a conventional cement sheath in a HPHT well in South Texas.
These results illustrate how both the completion and high-pressure fracture-stimulation processes lead to complete debonding of the cement sheath from the casing. These results are typical for HPHT wells when cemented with conventional cements. To help prevent this, operators tasked with constructing HPHT wells should more closely evaluate solutions for the life of the well, paying particular attention to a stress analysis on the set cement sheath.
LIFE-OF-WELL SOLUTION
A number of methods can be used to enhance the mechanical properties of a set cement sheath to help prevent damage and potential failure during the life of the well. With any of these methods, the ultimate goal is to create a more elastic cement sheath by lowering the Young’s modulus and increasing the Poisson’s ratio.
One option is to foam the cement using nitrogen. One way of describing the effect of the nitrogen in the set cement is that the nitrogen bubbles act like “cushions” to help absorb the change in stress created during operations on the well. Foamed cement has been used in HPHT wells in the Gulf of Mexico to good effect and in Texas on deep land wells for primary cementing since at least 1984.
Unfortunately, to create a foamed slurry at the density required to maintain well control in the HPHT wells of South Texas, the base slurry density would have to be more than 22 lbm/gal. It is technically possible to design cement slurries at such high densities; however, they become an impractical solution in this environment because of the extremely high viscosities created by first densifying the base slurry, then energizing that base slurry with nitrogen, further increasing the viscosity of the resulting foamed fluid.
Therefore, alternative means of enhancing the mechanical properties of the set cement sheath should be examined.
In recent years, it has been possible to modify the mechanical properties of set well cement with dry-blended additives, such as carefully selected elastomers. These additives are used to help make the cement more elastic than conventional cements and thus more able to withstand pressure cycles and stresses imposed on it without failing catastrophically. Other additive materials, such as fibers, are used to enhance the tensile strength of the set cement.

When designing HDEC, it is necessary to carefully select the correct ratio of mechanical-modification additives, heavy-weight additives and cement. HDEC are generally designed to possess lower values for Young’s modulus and friction angle and higher values for cohesion, tensile strength and Poisson’s ratio than conventional cements. Figure 2 illustrates the results observed when an HDEC is subjected to the same analysis as the conventional cement illustrated in Figure 1.
The overall changes to the cement’s mechanical properties resulted in a cement sheath that will maintain more than 80% capacity for the life of the well, dramatically better than results with a conventional cement.
To reiterate the concern related to high ECDs during placement of these heavy-weight slurries, all available tools should be considered when performing the cementing and casing design. Expandable liner hangers (ELH) have been successful in helping reduce the ECDs on production-liner jobs in South Texas. In Figure 3, the differences between an ELH and a conventional, mechanical liner hanger are highlighted. The key benefit of an ELH from a cementing perspective is the pre-expansion, larger flow area of the ELH, which creates less of a “choke point” in the flow path during the primary cement job than conventional liner hangers. This allows for greater pump rates to be achieved before maximum ECD restrictions in a wellbore are met. This translates to better mud removal, less chance that losses will be incurred, and all else being equal, a better quality of initial zonal isolation.

CASE STUDIES
The Yegua formation a key target reservoir in South Texas. Generally, when the Yegua is completed without any sand-control measures, the well requires periodic cleanout because of the accumulation of formation sand in the wellbore. Mechanical remediation involving gravel packs or vent screens and resin-coated fracture treatments have been used.
When the Yegua is completed without any sand-control measures, it can sometimes produce naturally without making formation sand; however, at some point, the bottomhole flowing pressure (BHP) might be drawn down to the extent where the critical-failure stress might be reached in the rock matrix and the reservoir collapses, potentially point loading the cement and casing and causing it to fail.
As noted previously, debonding and shear deterioration caused by high pressure differentials are also potential hazards that the cement sheath will face throughout its intended design life.
Operators have documented casing failures of this nature in South Texas HPHT fields. Casing failures occurred within six months to one year after the wells were put on production, leading to loss of production revenue, expensive remedial work, and even well abandonment.
One operator wishing to minimize the occurrence of such well failures considered all facets of their planned cementing operation for improvement opportunities. The cement bond logs of the failed wells in question before production had shown good zonal isolation; therefore, inadequate annular cement coverage was not considered to be the root cause. Instead, the mechanical properties of the conventional cement sheath were thought to be inadequate to withstand well completion and production operations. It was therefore decided to implement an advanced cement-job design approach to address this issue; an approach that considered the desirable mechanical properties of the cement sheath in addition to the necessary short-term cement slurry properties (i.e., gas migration prevention, fluid loss, 24-hour compressive strength, etc). The operator applied this approach, using HDEC, on 10 wells with success.
G #1 Well
A specialized finite element analysis-based diagnostic software package was used to assess the situation in the Cimarex G #1 well in Liberty County, Texas, to determine if a cement sheath of known mechanical properties would be expected to withstand planned well operations in that specific well. The conclusion was that a conventional, high-density cement sheath would likely fail under the loads imposed by planned well operations (Figure 1). Therefore, a more elastic HDEC, possessing the necessary mechanical properties, was designed that could be expected to withstand those operations without failure (Figure 2).

The G #1 wellbore geometry is provided in Figure 4.
The production interval was drilled with a 6 ½-in. bit at an inclination of 34.5° through the target formation sand, to a casing setting depth of more than 16,000 ft MD, with 17.5-lbm/gal OBM. Wireline logs were then run, including a multi-axis caliper. The caliper log estimated an average hole size of 7.92 in., with a couple of washed-out sections higher up the hole with IDs of 12 in. to 23 in. A tight margin existed between the pore pressure of 17.4 lbm/gal in this hole section and an estimated fracture gradient of 18.0 lbm/gal.
The BHST at TD was 296°F, and temperature-simulation software was used to calculate a BHCT of 240°F (Figure 5). Dynamic hydraulic-simulation software was then used to optimize the cement-slurry placement parameters so that the job design would be expected to control the zones with high pore pressure and, at the same time, not break down the formation. This work identified that the following fluid densities and volumes would be used: 50 bbl of 17.7-lbm/gal optimized rheology spacer (ORS) fluid incorporating suitable surfactants for OBM compatibility, followed by 117 bbl of 18-lbm/gal HDEC. The software indicated that these fluids should be able to be circulated into the wellbore safely at 3 bbl/min to achieve the planned TOC at 12,733 ft MD.
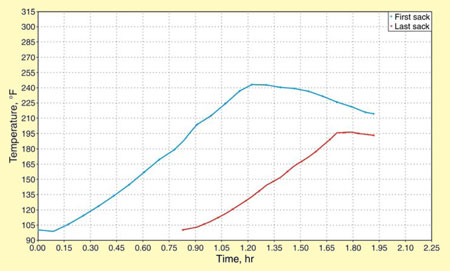

Properties of the HDEC selected are shown in Figure 6.
The 117 bbl of HDEC was mixed to density in a cement pump truck and transferred to two 100-bbl capacity batch mixers. Simultaneously, 50 bbl of ORS were also batch-mixed. These fluids were then pumped into the well as planned. The top plug was dropped, and the cement was displaced with 11.6-lbm/gal calcium chloride brine at 3.5 bbl/min. The plug was bumped after a displacement volume of 119 bbl and final circulating pressure of 5,970 psi. The bump pressure was then increased to 7,200 psi. The actual job chart is provided in Figure 7. Tank straps indicated full returns throughout the cement job with no mud loss.

An initial CBL (Figure 8 ) indicated good bonding and provided evidence that effective displacement efficiency was achieved during the cement job. The G #1 well was then completed and put on production. No remedial work was required, and no problems with well integrity have been reported.

BH #1 Well
The BH #1 well was drilled as an exploratory well in Hardin County, Texas, to test the Cook Mountain sand. This sand was found to possess economically productive pay, and a production liner was set. The well was drilled in a field where a high potential existed for production casing failures (collapsed pipe), caused by either formation subsidence or formation collapse occurring because of increased drawdown during well production. The BH #1 wellbore geometry is shown in Figure 9.

A 6 ½-in. production hole was drilled with 17.4-lbm/gal OBM. The estimated pore pressure at TD was 17.2 lbm/gal, and the fracture gradient at the 7 5/8-in. shoe was 18.0 lbm/gal.
The 6 ½-in. open hole was logged, and an average hole size of 6.5 in. was estimated from the caliper log. The planned TOC was 11,590 ft MD. As with the first case study, a tight margin between pore and fracture pressure existed. A 4 ½-in. production liner was therefore selected as the production string to reduce ECDs during the cement job while still being large enough to accommodate well completion requirements. An expandable liner hanger was also used to help reduce ECD during the running and cementing of the liner string.
Dynamic hydraulic-simulation software was again employed to help optimize pump rates and fluid rheologies for maximum mud displacement. From this work, it was decided to cement the well with 50 bbl of 17.7-lbm/gal ORS fluid, incorporating suitable surfactants for OBM compatibility, followed by 35 bbl of 18 lbm/gal-HDE cement to be pumped in the well at 3 bbl/min.
For this well, the HDEC also incorporated an in-situ gas-generating, anti-gas-migration additive because the simulation indicated that a severe gas-flow potential condition might exist in the well at job completion. HDEC properties are shown in Figure 10.

After the 4 ½-in. production liner was run to TD, circulation was established to condition the 17.4-lbm/gal OBM at a maximum rate of 3 bbl/min. At this time, only partial returns were noted. The HDEC slurry and ORS fluid were prepared in individual batch mixers. These fluids were then pumped at 3 bbl/min. The drill pipe dart was then dropped, and the cement was displaced at 3 bbl/min with 17.4-lbm/gal OBM. The plug was bumped after 178 bbl of displacement, at a final circulating pressure of 1,050 psi and bump pressure of 1,698 psi.
The operation of expanding the liner hanger was then conducted and was successful. There were partial mud returns for the entire cement job. The actual job chart is provided in Figure 11.

A cement bond log of the production liner indicated excellent bonding (Figure 12). The well was completed and put on production. No remedial work has been required on this well, and no problems with well integrity have been reported.

BEST PRACTICES

As noted previously, personnel in the southern Texas area have had the opportunity to use the diagnostic FEA software and deploy HDEC in numerous wells. They have developed a set of work methods and best practices for the successful application of this technology:
- While mechanical-property enhancing (MPE) admixtures can easily be added to cement systems, care should be taken that other slurry properties are not adversely affected. Some MPE admixtures have secondary effects that should be taken into consideration so that when the cement sets solid, the admixtures can provide the desired enhancement. For example, some MPE admixtures might, on their own, increase the rheology of a cement slurry and can also affect how the rheology is measured.
- Rheological measurements of HDEC in slurry form can be complicated by the size of the MPE admixture particles used and the clearance between the bob and sleeve of the standard rotational viscometer. In these cases, a special yield stress adapter (YSA) (Figure 13) is recommended. The YSA readily and accurately measures the yield stress of various fluids, such as particle-laden fluids. It is a special bob/sleeve combination that readily converts a Fann 35 viscometer to handle fluids with particulates, such as cement slurries with MPE admixtures, etc.
The design of the YSA is simple, but it is unique in its accuracy for measuring volume average shear rate versus stress data, as well as physically measuring the actual YP of a fluid system while using the standard Fann 35 viscometer equipped with a No. 1 spring. The geometries of the YSA bob (stator) and YSA sleeve (rotor) are designed so that particles of a wide range of densities can easily be volumetrically suspended during rheological measurement.
- When HDEC slurries are placed downhole at elevated temperatures, in common with other HPHT slurries, they can experience “thermal thinning.” Cement designers should be aware of this and guard against it, while still ensuring that the slurry does not exhibit any excessive viscosity that might inhibit mixing on the surface.
- Slurry stability is always critically important, and a well-simulation slurry stability test is performed on the cement slurry at HPHT conditions in an effort to detect any settling tendencies.
- The components of HDEC should ideally be dry-blended in the bulk plant and transferred on location.
Industry-recognized HPHT cementing best practices are also applied to help ensure that the chosen HDE sealant is placed in the wellbore annulus, where it is supposed to be, safely and efficiently. For example:
- When mixing slurry, extensive laboratory testing is done to help ensure that the slurry exhibits the right properties at surface and downhole conditions. Test conditions also reflect field-mixing technique, as some additives are sensitive to shear. For small volumes of cement slurry where a batch mixer is used to homogeneously mix the slurry, for example, the batch-mixing portion of the operation is usually simulated by stirring the slurry in a consistometer for the appropriate period at ambient temperature and pressure to impart an appropriate mixing energy.
- Fluid loss requires the use of a stirring fluid-loss cell in most HPHT situations to conduct the test using the actual temperature schedule. An API fluid loss of 70 cc or less in 30 minutes is acceptable. Most cement slurries tend to lose water to formations as the cementing operation is being carried out. Dehydration of the cement might result if the fluid loss is not controlled, and slurry movement can become difficult.
In cementing deep liners, the loss of fluid from a cement slurry can become a more serious problem than it is on long strings of casing. Because of little clearance between the liner and the wellbore, excessive loss of fluid from the slurry might cause bridging of the annulus and result in termination of the operation before the cement is in the desired position. In most cases, it is not necessary to completely prevent any loss of fluid from the cement slurry; rather, it is desired to control the amount of fluid that is lost.
- When dealing with gas migration, in many HPHT wells, pore pressure and fracture pressures are close to each other, which tends to increase the potential for gas migration. Gas can potentially enter the wellbore and flow up through or around the cement column from an overpressure zone to the surface or into a lower-pressured zone up the wellbore.
The likelihood that gas might flow up through the unset cement, if nothing is done to inhibit this flow, is determined by the gas flow potential (GFP). The GFP is used to determine the severity of the wells potential to experience annular gas migration problems. The GFP can be calculated from the following equation:
Preventing gas migration can be accomplished through slurry modification, job design changes, or a combination of both. To help prevent the formation of a gas channel in the unset cement, conventional slurries can be modified in five ways: decreasing volume losses, extending zero gel time, decreasing transition time, adding gas influx preventing materials, and/or increasing slurry compressibility.
To help prevent the formation of a gas channel in unset cement, the job design can be modified in four ways: decreasing effective column height, increasing overbalance pressure, interfering with the gellation process, and/or drilling a larger diameter hole.
- When dealing with job monitoring/execution, real-time job monitoring and data acquisition is extremely important for HPHT cement jobs. Parameters such as ECD, pressure, flow rate and fluid density can be captured by real-time data acquisition software at the rig site, allowing on-the-spot decisions to be made in the job execution to facilitate successful placement of cement.
- When dealing with compatibility testing, it is well known that the contamination of cement slurries with drilling fluids can cause severe problems, such as high viscosities, short thickening times, or failure of the cement slurry to develop sufficient compressive strength. Even the intermixing of the spacer or flush ahead of the cement slurry with the drilling fluid can, if incorrectly designed, possibly cause problems. It is therefore necessary that all fluids that can interact with each other downhole be compatible.
Compatibility testing of these fluids is done before a cement job, and if the fluids cannot be made compatible, job-design changes can be made, whereby the possibility of the two incompatible fluids coming in contact with each other is minimized.
- When dealing with mud removal, the tight pressure constraints found in HPHT wells in southern Texas mean that the desired density and rheological hierarchies are sometimes difficult to achieve without exceeding formation-fracture pressure. Therefore, the importance of other good drilling and cementing practices, such as conditioning the drilling fluid correctly, casing centralization, pipe movement when possible, and good spacer design all should be considered and implemented to increase mud-displacement efficiency.
CONCLUSION
In 1990, cement-sheath stress failure was identified as a leading cause of wells failing after they have been placed on production. In 1999, FEA-based software became available, as did a methodology that could be used to diagnose whether a cement sheath with certain mechanical properties might be expected to fail as a result of those loading conditions.
In 2003, the application of foamed cement to a HPHT well in the North Sea because of its superior mechanical properties and ability to better withstand stress failure was detailed. In 2006, workers described the application of high-density, mechanically modified cements in south Texas to solve HPHT well failures.
This technology has seen widespread application in South Texas. HDEC have been placed in wells in the area on 47 occasions to date. Of those 47 instances, the authors are aware of only one case of a wellbore in which HDEC was applied and the wellbore still failed. In that case, casing collapse was noted after significant sand production and the creation of a void space behind the cement, and point loading was suspected.
Acknowledgements: The authors thank the management of Cimarex Energy and Halliburton for their support and permission to publish this paper.
This article is based on “The Application of High-Density Elastic Cements to Solve HPHT Challenges in South Texas: The Success Story,” SPE 122762, copyrighted by SPE and presented at the 2009 SPE Annual Technical Conference and Exhibition, New Orleans, La., 4–7 October.
References:
Benge, O., McDermott, J., Langlinais, J., and Griffith, J. 1996. Foamed Cement Job Successful in Deep HPHT Offshore Well. Oil & Gas Journal, March.
Biezen, E. and Ravi, K. 1999. Designing Effective Zonal Isolation for High-Pressure/High-Temperature and Low Temperature Wells. Paper SPE 57583 presented at the SPE/IADC Middle East Drilling Technology Conference, Abu Dhabi, United Arab Emirates 8–10 November. DOI: 10.2118/57583-MS.
Bosma, M., Ravi, K., vanDriel, W., and Schreppers, G. 1999. Design Approach to Sealant Selection for the Life of the Well. Paper SPE 56536 presented at the Annual Technical Conference and Exhibition, Houston, Texas, 3–6 October. DOI: 10.2118/56536-MS.
Bozich, M., Montman, R., and Harms, W. 1984. Application of Foamed Portland Cement to Deep Well Conditions in West Texas. Paper SPE 12612 presented at the SPE Deep Drilling and Production Symposium, Amarillo, Texas, 1–3 April. DOI: 10.2118/12612-MS.
Continental Shelf Operations Notice No. 59 Department of Energy. 1990. Applications for Consent to Drill or Reenter High-Pressure High Bottomhole Temperature Exploration and Appraisal Wells: Supplementary Information to be Supplied in Addition to That Required by CSON 11. London, England, May.
Darbe, R., Gordon, C., and Morgan, R. 2008. Slurry Design Considerations for Mechanically Enhanced Cement Systems. Paper AADE-08-DF-HO-06.
Ellis, V., Cormier, G., Adams, G., and Santos, F. 2005. Fracturing for Sand Control: Screenless Completions in the Yegua Formation. Paper SPE 96289 presented at the Annual Technical Conference and Exhibition, Dallas, Texas, 9–12 October. DOI: 10.2118/96289-MS.
Goodwin, K. and Crook R. 1990. Cement Sheath Stress Failure. SPE Drill Eng 7 (4): 291–296. SPE-20453-PA. DOI: 10.2118/20453-PA.
Heathman, J. and Beck, F. 2006. Finite Element Analysis Couples Casing and Cement Designs for HT/HP Wells in East Texas. Paper SPE 98869 presented at the IADC/SPE Drilling Conference, Miami, Florida, 21-23 February. DOI:10.2118/98869-MS.
Hoover, E. and Trenery, J. 2008. High-Performance WBM Optimizes Drilling Efficiency In Demanding Vicksburg Wells. The American Oil & Gas Reporter, August.
Ravi, K. and Bosma, M. 2003. Optimizing the Cement Sheath Design in HPHT Shearwater Field. Paper SPE 79905 presented at the SPE/IADC Drilling Conference, Amsterdam, The Netherlands, 19–21 February DOI: 10.2118/79905-MS.
RP 10B-2/ISO 10426-2, Recommended Practice for Testing Well Cements, first edition. 2005. Washington, DC: API.
Schenk, C. and Viger, R. 1995. Western Gulf Province (047). 19–22.
Strickler, R. and Solano, P. 2007. Cementing Considerations for Casing While Drilling Operations: Case History. Paper SPE 105413 presented at the SPE/IADC Drilling Conference, Amsterdam, The Netherlands, 20–22 February. DOI: 10.2118/105413-MS.
Sutton, D., Sabins, F., and Faul, R. 1984. Preventing Annular Gas Flow. Oil & Gas Journal 82: 84–92.
Sweatman, R., Kelley, S., and Heathman, J. 2001. Formation Pressure Integrity treatments Optimize Drilling and Completion of HPHT Production Hole Sections. Paper SPE 68946 presented at the European Formation Damage Conference, The Hague, Netherlands, 21–22 May. DOI: 10.2118/68946-MS.
yo
Your theme about this topic is very entertaining. I have to opt for the research paper writing services to buy essays and already written essay about this topic, because that is the good way.
Sehr gehte Herren und Damen
Ich brauche bitte ein export preis für der High pressure – High temperature ( HPHT ) Consistometer
Mitt freundlchen grußen
Khalid Hassan
– High pressure – High temperature ( HPHT ) Consistometer.
( Max . temp.450 º F m , Max . pressure 25000 psi , 220 V/50 HZ )
including the following :-
– Complete slurry cups assembly , Qty. (4)
– Calibration device with Calibration oil , Qty .1 set .
– Spare parts for two years , Qty . 1 set .
– Training ( Two ) persons for installation and operation for ( Two ) Weeks
– Origin ( USA , Germany , France , UK , Japan )
Delivery ( 4) four Months from Date of assignment .